Nano-sized clay stabilizers show promise in protecting formation permeability
Clay swelling is a well-known source of formation damage in hydrocarbon reservoirs. In conventional production situations, clay swelling at the near wellbore area can hinder drilling, completions, and workover activities. In enhanced oil recovery operations, clay swelling or fines migration can impair the permeability of the formation.
Numerous research studies over the past 50 years have contributed to the industry’s understanding of how clay stability is impacted by the composition of aqueous fluids in the well. Previous works have shown how changes to salinity, pH, temperature, and flowrate all lead to clay swelling and migration in sandstone reservoirs.1, 2, 3, 4, 5, 6 Clay swelling inside the reservoir reduces the free area available for the passage of fluids, which restricts fluid flow. Released clay particles get carried with the fluid through the reservoir’s complex network of pores, where they can block the pore throats, reduce permeability, and slow production flow rates.
Clay stabilizers are used widely to prevent agglomeration, pore blocking, and flow impairment. Common stabilizers include chlorides of sodium, potassium and zirconium; hydroxides of sodium, calcium and aluminum; salts of aluminum, zirconium and quaternary ammonium, and cationic organic polymers7. Most are often inadequate due to their inability to migrate through smaller pores, difficulty in handling and disposal, deleterious environmental effects, and high cost.
These challenges prompted an investigation into the use of nanoparticles to stabilize clay particles and prevent flow impairment. Nanoparticles, which have at least one dimension less than 100 nanometers (nm), exhibit unique chemical properties compared to particles on the micro scale or larger. As particle size decreases, more atoms are exposed on the surface, thus enhancing surface activity. As the size of a sphere decreases from the micro to the nano scale, the surface area increases by 1,000 times in comparison to the volume. Nano-sized particles are expected to easily pass through reservoir pores and pores throats, which are several microns in size.
This article reviews a study that investigated using colloidal nanoparticles to prevent clay-related flow impairment in the reservoir. Silica-based nanoparticles were selected because of their abundant availability, lower production cost, and non-toxicity compared to commonly used clay stabilizers.
EXPERIMENTAL PROCEDURe
Material. Silica-based inorganic colloidal nanoparticles possessing a net positive surface charge (cationic nanoparticles) and negative surface charge (anionic nanoparticles) were selected for these tests. Separate brine solutions were prepared by mixing sodium iodide and sodium chloride, respectively, in deionized water.
Core materials and preparation. Berea sandstone core samples, each with a diameter of 1.5 in. (3.8 cm) and length of 4 in. (10 cm), were used as a model rock to test the transport behavior of nanoparticle formulations and their role in clay stabilization. Cores were cleaned by running deionized water through them, and thoroughly dried in the oven at 100°C (212°F). Dried cores were CT scanned to visualize core internals and to ensure they were free of cracks and defects.
Coreflood test apparatus. The coreflood setup consisted of a hydrostatic core holder, a vacuum pump, pumps for injecting fluids, an overburden pressure pump, floating piston accumulators for brine and nanoparticle dispersions, and an automatic sample collector for collecting the eluting samples at regular intervals, Fig. 1. The core’s temperature and pressure at the inlet and outlet were also recorded.
Particle size distribution and charge. Nanoparticle stability in a dispersion strongly depends on surface charge. Therefore, the zeta potential for nanoparticles in the brine dispersion was measured using a Malvern ZetSizer Nano ZSP instrument. This same instrument was used to collect dynamic light-scattering measurements for particle size distribution of the nanoparticles.
Bottle tests. Bottle tests conducted on a swellable montmorrilonite clay aimed to understand the effect of nanoparticles in stabilizing clay particles and mitigating swelling behavior. Zeta potential and particle size measurements were also conducted on these samples.
STUDIES AND RESULTS
Nanoparticle stability and size. Nanoparticle stability in the dispersion is crucial for effective transport through the core. A poorly prepared dispersion tends to settle over time by particle aggregation brought on by electrostatic charge imbalance. Separate cationic and anionic nanoparticle dispersions were prepared and visually observed for their stability. The dispersions were stable for several months, with no visible sign of precipitation.
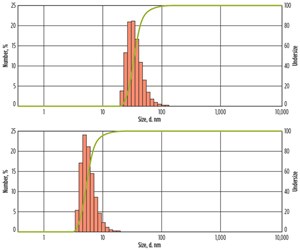
Past research has shown that for a stable dispersion, nanoparticles should have zeta potentials greater than +30 medium voltage (mV) or smaller than –30 mV. The zeta potentials of the cationic and anionic nanoparticles in this study were +38.2 mV and –36.0 mV respectively, which translated to excellent stability of these two nanoparticle dispersions.
Dynamic light scattering measurements of the nanoparticle dispersions confirmed that the particles are much smaller than the typical pore and pore throat sizes in most reservoirs, which measure several microns across, Fig. 2.
Nanoparticle stability inside the rock. Unhindered transport of nanoparticles through porous media is important for their ability to successfully stabilize clay inside the reservoir. Nanoparticles in the dispersion encounter more resistance compared to the carrier phase when injected into the core. Depending upon their affinity to rock, neighboring nanoparticles, and the carrier phase, they might aggregate at the rock face and form filter cake without entering the rock or successfully flowing into the core. They could also aggregate in the pores and block pore throats, which can severely damage reservoir permeability. Therefore, studying nanoparticle transport in porous media during the core flooding setup is essential.
Prior to injecting nanoparticles into the core, a quick test was performed, in which nanoparticle dispersions were injected through a filter with 200-nm pores. The dispersions of both anionic and cationic nanoparticles passed through the core without leaving any residue on the filter, suggesting efficient transport in the core.
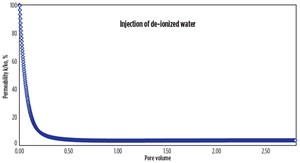
Core flood experiments. A series of flow experiments evaluated the effect of separate cationic and anionic nanoparticle treatments on the clay stability inside the core. A shock experiment similar to a previous experiment6 was conducted to simulate clay-related flow impairment in the laboratory. Berea sandstone core was dried, weighed, and placed in the core holder, after which an overburden pressure of 1,000 psi was applied and the core was vacuumed at room temperature. A 6-weight-percent (wt%) sodium iodide (NaI) solution was then injected into the core, and the permeability was measured, Table 1. To mimic formation damage, the NaI injection was stopped and deionized water was injected. The change in salinity resulted in a rapid increase in differential pressure and a 99% reduction in permeability due to clay migration and blocking of pores and pore throats, Fig. 3.
Rock treatment was initiated immediately after the deionized water injection by injecting 0.1 wt% of anionic nanoparticle dispersed in deionized water, Table 1. The injection of anionic nanoparticle resulted in a rapid increase in pressure, which may have been due to the previously injected deionized water causing a sodium ion exchange with the clay particles and initiating clay migration. The anionic nanoparticle treatment could not pass through the already restricted pores and pore throats.
Rock treatment was initiated immediately after the deionized water injection by injecting 0.1 wt% of anionic nanoparticle dispersed in deionized water, Table 1. The injection of anionic nanoparticle resulted in a rapid increase in pressure, which may have been due to the previously injected deionized water causing a sodium ion exchange with the clay particles and initiating clay migration. The anionic nanoparticle treatment could not pass through the already restricted pores and pore throats.
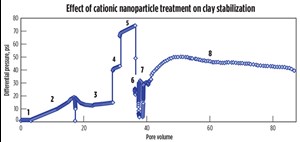
In the next core flood, a berea core saturated with 1-wt% NaI solution was squeeze treated with a cationic nanoparticle dispersion, also in a 1-wt% NaI solution. After the rock treatment with cationic nanoparticle, deionized water was injected into the core. Treatment of the core with the cationic particles substantially reduced the permeability impairment and the pressure drop was much smaller compared to the core flood experiment, Fig. 4. This behavior could be the result of electrostatic adsorption of the positively charged nanoparticles on the negatively charged faces of the lamellar clay surfaces (Fig. 5), which served to minimize the clay’s interaction with the fresh water. A total of 87 pore volumes of fluids were injected into the core in sequence, of which 68 pore volumes were deionized water. The pressure profile at the end of 87 pore volumes still showed a decreasing trend, Fig. 4.
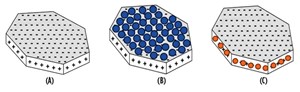
In the final core flood experiment, a Berea sandstone core sample was prepared in the same manner as previous tests, but with a 1-wt% sodium chloride (NaCl) solution injected into it at room temperature. The core was treated with anionic nanoparticle dispersion in 1-wt % NaCl solution. A comparison of core permeability before and after nanoparticle treatment showed little change. Permeability was 48 millidarcy (mD) before treatment, and it dropped slightly to 46 mD after the treatment. This reduction may be a result of the adsorption of the negatively charged nanoparticle on to the positively charged edges of the clay particles, Fig. 5.
Deionized water was then injected into the core, and no flow impairment was registered up to 2.8 pore volumes. However, the pressure across the core quickly increased upon further water injection, and clay particles were seen in the eluting samples. One possible explanation for no initial permeability impairment could be that the adsorption of anionic nanoparticles on the positively charged edges of the clay particles prevented intercalation of water molecules and ions into the clay laminated structures. However, the electrostatic attraction forces that initially keep the negatively charged nanoparticles on the clay edges might not be strong enough to withstand exposure to deionized water. This would result in eventual clay migration and blocking of pores and pore throats, causing the observed rapid increase in differential pressure across the core.
Bottle test results for stabilizing swellable clay. Montmorillonite, a swellable clay commonly found in sandstone formations, was dispersed in deionized water with and without the anionic nanoparticles. In the samples without nanoparticles added, almost all the clay particles precipitated and formed a thick layer. The addition of the nanoparticles caused the clay particles to form a much thinner precipitate layer and stabilize, leaving fine clay particles in the deionized water.
The anionic nanoparticles were then tested for their ability to stabilize clay particles at high pH conditions, using processed water containing various monovalent and divalent ions. Visual observations showed that the nanoparticles dispersed the clay quite efficiently, and the clay remained suspended in water at concentrations as low as 150 parts per million for at least two weeks. However, the zeta potential of the clay-nanoparticle system remained the same, and zeta potential did not vary with the concentration of nanoparticles. Once again, a possible explanation is that the anionic particles stabilize the clay by adsorbing on to the positively charged edges. Because the edge surface area is much smaller than the negatively charge face surface area, this would serve to increase the overall negative charge of the clay particle.
CONCLUSIONS
These laboratory tests demonstrated that the stability of clay particles is significantly improved by adding inexpensive, environmentally benign nanoparticle colloids to the aqueous fluid system. Improved permeability was observed after treating the sandstone core samples with nanoparticles, although the degree of this improvement depended on the surface charge of the nanoparticle.
During coreflood shock experiments in which deionized water was injected to simulate clay-related flow impairment, the cationic silica nanoparticles reduced clay migration and significantly lowered flow impairment through a total of 87 pore volumes of fluid injected into the core, the majority of which were deionized water. The anionic nanoparticles demonstrated no flow impairment during the first two to three pore volumes of deionized water injection. A rapid increase in differential pressure across the core, indicated clay migration and pore blocking.
These results suggest two different stabilization mechanisms at work. A possible mechanism for the reduction in permeability impairment is the attachment of positively charged nanoparticles to the negatively charged clay surfaces, which serves to pin clay particles in the pore surfaces. Negatively charged nanoparticles attach to the positively charged edges of the clay particles, which makes up a smaller portion of the clay’s surface area. The electrostatic attraction forces between the anionic nanoparticles and the clay’s edges are not strong enough to prevent clay migration into the pore throats.
However, the adsorption of anionic particles seems to help prevent water molecules and ions from intercalating into the clay laminated structures and causing clay swelling. This was confirmed in bottle tests that showed the anionic nanoparticle’s efficacy in stabilizing montmorillonite clay and suppressing clay swelling.
While this initial research shows promise in using nanoparticles to improve clay stability, it is merely a first step. Future research should focus on testing combinations of cationic and anionic nanoparticles in different ratios, to determine if their individual contributions to clay stability—namely, swelling suppression from the anionic particles and clay pinning from the cationic particles—result in a synergistic performance improvement that promises to benefit all well construction and production enhancement operations.
References
- Gray, D. H. and R. W. Rex, “Formation damage in sandstones caused by clay dispersion and migration,” paper presented at the 14th National Conference on Clays and Clay Minerals, London, Chevron Research Co., 1966, pp 355.
- Mungan, N., “Permeability reduction through changes in pH and salinity,” Journal of Petroleum Technology, December 1965.
- Mungan, N., “Permeability reduction due to salinity changes,” J. Cdn. Pet. Tech., 1968, pp 113.
- Jones, Jr., F., “Influence of chemical composition of water on clay blocking of permeability,” Journal of Petroleum Technology, April 1964.
- Hewitt, C. H., “Analytical techniques for recognizing water-sensitive reservoir rocks,” J. Pet. Tech., August 1963, pp 18–813.
- Khilar, K. C. and H. S. Fogler: “Water sensitivity of sandstones,” SPE Journal, pp. 23, 55–64, SPE paper 10103, http://dx.doi.org/10.2118/10103-PA, 1983.
- El-Monier, I. A. and H. A. Nasr El-Din, “A new environmentally friendly clay stabilizer,” Society of Petroleum Engineers, 2010.
- Agrawal, D. K., O. V. Kuznetsov, R. Suresh, J. Welch, and V. N. Khabashesku, “Environment-friendly colloidal nanoparticles for stabilizing clays within subterranean formations,” SPE paper 181641, https://doi.org/10.2118/181641-MS, 2016.
- Well control considerations for a global geothermal industry (December 2024)
- Sustainability: The relationship between upstream operations and blue hydrogen production (November 2024)
- Novel approach of recovering energy from high-pressure multiphase streams (November 2024)
- What's new in production: Banking crisis (October 2024)
- General Bond Log Interpreter completes interpretation, based on standardized rules and data formats (September 2024)
- EY says U.S. E&P fared well in 2023 while ESG reporting increased further (September 2024)